Lecture 14 and 15Transport
|
Structure of mitochonrion
A new view of mitochondrial structure.
Transport systems in the inner mitochondrial membrane
The mitochondrial metabolite transport systems were recognized in Mitchell's original paper as an essential component of the chemiosmotic circuit. The main carrier systems were first characterized in Chappell's lab, the earliest ones being the phosphate/OH- exchange system (the phosphate transporter), and the ADP/ATP- exchange system (the adenine nucleotide transporter). Subsequently, the transporters for citrate and isocitrate, malate and succinate, and 2-oxoglutarate (a-ketoglutarate) were quickly demonstrated. Inhbition of transport by specific inhibitors which also inhibited substrate utilization by mitochondria demonstrated that transport was by specific carrier.
The demonstration of the mitochondrial transport systems (and the wider context of the chemiosmotic controversy) stimulated an explosive growth in the search for transport systems in bacteria, chloroplasts, the cell membrane, and in other intracellular organelles.
The transport of ATP, ADP and phosphate across the mitochondrial membrane
The synthesis or hydrolysis of ATP by intact mitochondria occurs in the matrix space, because the catalytic sites are on the F1 head of the ATP synthase lollipop, which projects on the matrix side (N-phase) of the membrane. Nevertheless, mitochondria are able rapidly to hydrolyse externally added ATP, and to synthesise ATP outside from added ADP and phosphate. This is possible because of two transport systems which allow these metabolites to cross the membrane:
- The adenine nucleotide transporter.
This enzyme catalyses the exchange of ATP for ADP across the inner mitochondrial membrane. The charges on the substrates are such that ATP carries a net excess charge of -1 compared to ADP.

The exchange reaction is therefore electrogenic, and driven by the electrical component (Dy) of the proton gradient, so that entry of ADP and exit of ATP are favored.
In, or N-phase
Out, or P-phase
The thermodynamics of this process, are discussed in more detail here.
- The phosphate transporter.
The phosphate transporter was the first of the mitochondrial metabolite transport systems to be demonstrated (Chappell and Crofts, 1965). The enzyme catalyses the net transfer of H3PO4 across the membrane in a neutral process. Since the predominant ionic species present at neutral pH is H2PO4-, the mechanism must involve either exchange of H2PO4- for OH-, or cotransport of H2PO4- with H+.
H2PO4-L + OH-R <==> H2PO4-R + OH-L
or
H2PO4-L + H+L <==> H2PO4-R + H+R
The exchange allows the chemical component of the proton gradient (-ZDpH) to drive uptake of phosphate into the mitochondrion.
The net effect of these two transport processes is that the proton gradient drives the concentration of ADP and phosphate inside the mitochondrion, and the export of ATP, at the expense of 1H+/ATP synthesized.
More general aspects of the thermodynamics of transport are covered here.
Metabolite transport
Mitochondria have diverse metabolic functions, which vary from tissue to tissue. In liver mitochondria, a wide variety of externally added substrates can be processed by the mitochondrial metabolic machinery. The enzymes are predominantly in the matrix, requiring that substrates be transported across the mitochondrial inner membrane. The transport is catalysed by a superfamily of proteins, which show strong sequence homology across the whole spectrum of the eukaryote world, but no obvious prokaryote precursor. The metabolite transporters generally catalyse neutral exchanges, but some are electrogenic, or involve H+ cotransport or OH- exchange, so that the transport equilibrium is determined by the proton gradient.
The swelling of mitochondria in the ammonium salts of weak acids or metabolites
The transport activities for the metabolite systems were first demonstrated by use of a simple and elegant technique introduced by Brian Chappell. The technique depends on the following:
- Mitochondria behave as perfect osmometers; they respond to changes in osmotic pressure of the suspending medium by shrinking (or swelling) as water flows across the membrane to compensate for the difference in activity when the osmolytes are added to (or removed from) the external medium.
- The mitochondrial membrane is impermeable to small ions such as H+, K+, Na+, NH4+, Cl-, NO3-, etc.
- The mitochondrial membrane is permeable to small uncharged molecules such as O2, CO2, N2, 3-C or 4-C sugars, etc.
- Although the membrane is impermeable to small ions (item 2), and therefore to acetate-, and NH4+, it is permeable to acetic acid, and to NH3, which are neutral small molecules (item 3).
As a consequence of these permeability properties, mitochondrial will not swell in NH4Cl, or in K-acetate, because the membrane is impermeable to the ions. However, they will swell if suspended in NH4-acetate, because the neutral forms can cross the membrane.
In the picture below, mitochondrial swelling was measured in a simple light-scattering photometer. On addition of aliquots of mitochondrial suspension to distilled water, or 100 mM of the salts shown (pH adjusted to 7.0), after decreasing to an initial level due to absorption of light by the added mitochondria, the transmission increased as the mitochondria swelled; very rapidly in water, very slowly in KCl, K-acetate or NH4Cl, rapidly in NH4-acetate:
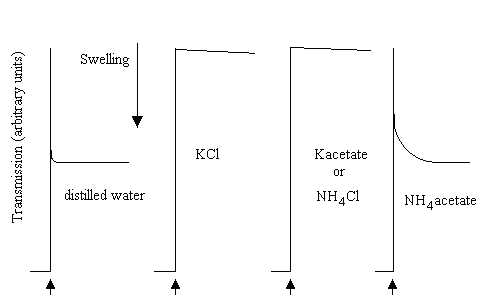
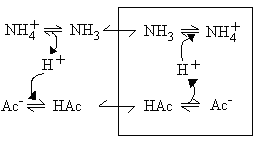
In similar experiments, mitochondria were added to the NH4-salts of various mitochondrial substrates:
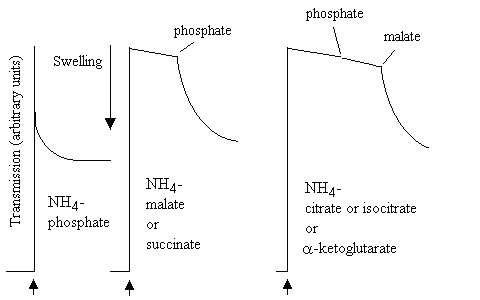
The mitochondria swelled rapidly in NH4-phosphate, but not in the other NH4-salts. However, on addition of catalytic amounts of K-phosphate to the malate or succinate experiment, or of K-phosphate and K-malate to the citrate, isocitrate or a-ketogltarate experiment, rapid swelling was observed. The results were interpreted as shown in the scheme below:
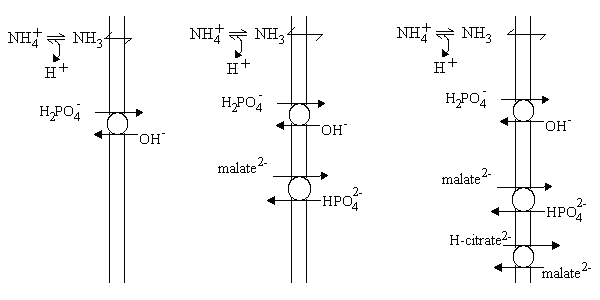
- Left: The presence of a phosphate transporter allowed H2PO4- to exchange for OH- across the membrane. This effectively allowed phosphate to cross the membrane as if it were a weak acid, so the situation was as for acetate.
- Center: The malate2- - HPO42- exchange transporter allowed malate to enter, but only if a catalytic amount of external phosphate was present to allow rapid turn-over of the phosphate transporter. Effectively, the activity of the two carriers allows malate2- to enter in exchange to 2OH-.
- Right: The citrate transporter allowed H-citrate2- (one of the three carboxylate groups is protonated) to enter in exchange for malate2-, but this could only occur rapidly if sufficient external malate and phosphate were present to allow rapid turn-over of their carriers. Effectively, citric acid enters as a neutral species (or in exchange for 3OH-).
In each case, the carriers allow the metabolites to enter as the neutral species; the anion exchanges for an equivalent number of OH- charges, and this allows an equivalent number of NH3 molecules to enter and be protonated to NH4+, allowiing net entry of the NH4-salt, and swelling of the mitochondria.
Family, sequences, structure
The family of proteins which includes the five members discussed above (phosphate transporter, adenine nucleotide translocator, tricarboxylate transporter, malate transporter, 2-oxoglutarate transporter) has been extensively studied in recent years. There are many sequences available, of which a sample can be found at this link.
The family also includes an interesting protein which is active in the mitochondria from bown adipose tissue. "Brown-fat" mitochondria are uncoupled,- their expression is thought to allow for the warming up of hibernating animals, by generation of heat. The mechanism of heat generation involves activation of the uncoupling protein, which acts as a proton carrier.
The ancestry of the mitochondrial tranporter family is obscure. Recently, the genome sequencing project on Rickettsia has shown a protein with sequence similarity to the ADP/ATP translocator of plant mitochondria. This makes it likely that the mitochondria were derived from an ancestor common to Rickettsia, which is an endosymbiotic bacterium, and that the ADP/ATP translocator came from this source. The ADP/ATP translocator is sufficiently different in sequence from the other metabolite transporters (phosphate, citrate/isocitrate, malate/a-KG, brown fat uncoupling protein, carnitine/acyl carnitine, etc.) that this ancestry cannot be generalized to cover all the mitochondrial transporters. It is possible that this latter set represent a specifically eukaryotic invention to facilitate integration of the mitochondrial endosymbiont into the metabolism of the host.
No tertiary structure for a mitochondrial transport protein is yet available. The mitochondrial family of metabolite transporters seem to have a conserved secondary structure, with three well defined hydrophobic spans which are likely tranmembrane helices, and a fourth span with hydrophobic and amphipathic character, which might also be transmembrane. There has been much speculation about the role of this and other amphipathic spans in the transport mechanism.
Ionophores, and transport of cations by mitochondria
Early evidence for the chemiosmotic hypothesis came from studies of ion transport by mitochondria. Three types of transport were studied:
Transport of bivalent cations
When a proton gradient is generated by coupled electron transfer or ATP-hydrolysis, mitochondrial take up Ca2+ from the external medium. The Ca2+-uptake is catalysed by a Ca2+ carrier in the inner membrane which exchanges Ca2+ for H+ (net electrogenicitiy, 1 + charge). When phosphate is also present, a net uptake of calcium phosphate occurs, which precipitates inside the mitochondria as hydroxyapatite, visible as dense granules in electron micrographs. If a weakly acid anion like acetate is present in the suspending medium, calcium acetate is accumulated, and the mitochondria swell osmotically because of the increased soluble salt inside.
Transport of Ca2+ plays a physiological role in those cell processes which are activated or inhibited by changes in Ca2+ activity in the cytoplasm. The mitochondrial transport has a Km in the physiological range, so uptake buffers the internal [Ca2+].
Mitochondria will transport Mn2+ or Sr2+ in place of calcium.
Role of mitochondrial permeability in programmed cell death (apoptosis)
Apoptosis is the mechanism by which the body rids itself of damaged cells. It plays a prominent role in protection against cancer. Over the last few years, it has become apparent that mitochondria are of critical importance in the apoptosis process. Proteins that control the survival or death of cells are specifically associated with the mitochondrial outer membrane, and permeability changes across the mitochondrial inner membrane, probably associated with Ca2+ transport and mitochondrial swelling, and the release of proteins, particularly cytochrome c, from the inter-membrane space, play an early role in the deciding the fate of the cell.
Apoptosis links
A brief tutorial on apoptosis by Dr. Chris McBride
A summary of mitochondrial involvement in apoptosis.
Another nice summary of apoptosis.
Proceedings of a recent workshop on apoptosis.
More on the role of mitochondria in apoptosis.
Ion transport catalysed by ionophores
Characterization of the mechanisms of ionophores played a crucial role in our understanding of the proton gradient, and its role in chemiosmotic coupling. Four ionophoric mechanisms have proved particularly useful:
Valinomycin
Valinomycin catalyses the transprt of K+ (and other monovalent cations of similar radius like Rb+ or Cs+, but not Na+) as a charged species across biological membranes (or hydrophobic phases in general). Transport is by a carrier mechanism. As a consequence, the electrochemical potential for the transported species is equal across the membrane, and the equilibrium condition applies. 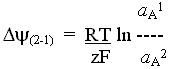
The distribution of ions (usually isotopically labelled Rb+ is used) between inner and outer phases in the presence of valinomycin has been a favorite method for measuring membrane potential.
As a result of this mechanism, mitochondria will take up K+ at the expense of the proton gradient driven by coupled electron transfer or ATP hydrolysis.
In the presence of a transportable anion (phosphate), or the anion of a permeable weak acid (eg. acetate), the mitochondria swell as they respond osmotically to the accumulation of salt.
In the presence of excess K+, the electrical component (Dy) of the proton gradient is collapsed, at least during the time taken to accumulate a substantial K+ gradient.
Nigericin
Nigericin catalyses the neutral exchange of monovalent cations across biological membranes, with a broad specificity. If a single cation predominates, the cation is exchanged for H+. As a consequence, when nigericin is added to mitochondria in the presence of excess K+, the -ZDpH component of the proton gradient is collapsed. The mechanism of nigericin is discussed in greater detail here.
Gramicidin
Gramicidin catalyses an electrogenic flux of small monovalent cations, including protons, across biological membranes through a pore formed by two molecules end-to-end spanning the membrane. The gramicidin pore is much studied, and is discussed in greater detail in the context of proton conduction.
As a consequence of the mechanism, gramicidin acts as an uncoupling agent. In the presence of excess monovalent cation (such as K+), the transport of K+ is favored over the leak or H+, and gramicidin will catalyse K+-uptake by mitochondria, similar to that seen with valinomycin.
Protonophores
Mitchell proposed that uncouplers act by short-circuiting the proton gradient,- acting as proton conductors. This protonophoric activity has been much investigated, and the demonstration of a strong correlation between protonophoric activity in artificial membrane systems, and uncoupling activity, was a strong piece of evidence favoring the chemiosmotic hypothesis. The mechanism Mitchell proposed is illustrated here for the classical uncoupler 2,4-dinitrophenol.
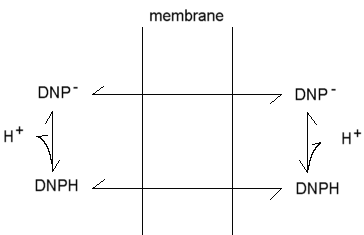
The phenol is weakly acid, and can exist in neutral and anionic forms. Because of the delocalization provided by the extended p-orbital, the charge is distributed over a sufficient volume to allow the anion to be weakly lipid-soluble. As a consequence, both neutral and charged forms can cross the membrane, leading to a cycle of protonophoric activity. This general mechanism describes the action of a wide variety of uncoupliing agents.
A nice set of Chime tutorials on Pores - channels - transport, with examples from known crystallographic structures of porins, toxins, channels and carriers (from Dr. P. Bergman, University of Hamburg, Dept. of Biology).
Metabolic communication between cytoplasm and mitochondrial matrix
The integration of the eubacterial metabolism of the mitochondrion and the metabolism of the host cell has led to a sharing of many metabolic pathways, and a traffic in metabolites across the mitochondrial membrane, catalysed by the metabolite transport systems. Some examples are given below:
Gluconeogenesis
The lactate returned to the liver from production in the muscle under conditions of high demand, is recycled back to glucose, using the energy from oxidation of a fraction of the lactate. The pathway is called gluconeogenesis, and the cell uses many of the enzymes of glycolysis, operating in the reverse direction. To drive the reaction in reverse, the cell increases the concentration of the input substrate, phosphoenol pyruvate (PEP), and bypasses the kinase reactions by which glucose is "activated" (which have a high -ve DGo' when operating in the direction of glycolysis) by using phosphatases. In order to overcome the relatively high -ve DGo' of the pyruvate kinase reaction, the cell uses enzymes in the mitochondrial matrix to allow production of PEP via carboxylation of pyruvate to oxaloacetate (OAA), conversion to malate, export to the cytoplasm, conversion back to OAA, and decarboxylation with phosphorylation to generate PEP. This series of reactions allows the free energy from hydrolysis of two ATP molecules to be used to convert pyruvate to PEP.
Import of reducing equivalents from cytoplasmic NADH
The mitochondrial membrane is impermeable to nicotinamide adenine dinucleotides (NAD+, NADH, NADP+, NADPH); nevertheless, the NADH generated in the cytoplasm by glycolysis is oxidized by the repiratory chain. This is achieved by two main pathways, the glutamate/aspartate shuttle, and dihydroxyacetone phosphate (DHAP) shuttle, which are used predominately in the liver and muscle respectively.
- The glutamate /aspartate shuttle
This pathway uses the mitochondrial metabolite transport systems as shown below:
The shuttle uses the a-ketoglutarate (a-KG)-malate exchange transporter, and the glutamate-aspartate exchange transporter, malate dehydrogenase to reduce oxaloacetate (OAA) to malate, using NADH outside (and the reverse reaction inside), and a transaminase enzyme to exchange the amino group between the glutamate/OAA and a-KG/aspartate pairs. An important feature of this mechanism is the role of the proton gradient in increasing the level of reduction of the mitochondrial NAD+/NADH couple. The glutamate/aspartate carrier catalyses an electrogenic reaction, because glutamic acid is exchanged with aspartate anion. The net effect is that the redox poise of the NAD+/NADH couple is displaced by a DE' = Dp.
- The DHAP shuttle
This pathway does not involve metabolite transport. Instead, it depends on the activity of two different glycerol-3-phosphate dehydrogenase enzymes, one using NAD/NADH couple, and the other, an FAD-linked membrane bound enzyme, which reduces the ubiquinone pool. The pathway for oxidation of cytoplasmic NADH is shown below:
Export of 2-C fragments for fatty acid synthesis
Acetyl-CoA is the substrate for fatty acid synthesis, but the production of acetyl-CoA occurs predominantly in the mitochondria matrix. Three main sources of acetyl-CoA are:
- Pyruvate dehydrogenase
- The breakdown of fatty acids
- Catabolism of ketogenic amino acids
The mitochondrial membrane is impermeable to acyl-CoA molecules, so the synthesis of fatty acids depends on a mechanism for export of 2-C fragments. A possible mechanism is shown below. The pathway depends on the activity of the citrate/malate exchange transporter, and formation of citrate from OAA and acetyl CoA inside (right) using the condensing enzyme, and production of acetyl CoA and OAA outside using ATP-dependent citrate lyase.
G protein-coupled receptors
The G protein-coupled receptors (GPCRs) are an important family of membrane proteins, including rhodopsin, in which binding of an affector molecule (or absorption of light) stimulates a cascade of enzymes linked to GTP/cyclic-GMP.
"The superfamily of GPCRs are integral membrane proteins. Their sequences show seven hydrophobic domains, commonly interpreted as seven transmembrane helices. GPCRs are found in a very wide range of species, and are always involved in signalling from outside the cell to inside the cell. Most GPCRs detect a periplasmic small molecule or a peptide
which upon binding elicits a response felt by the heterotrimeric G protein at the cytosolic side. Some GPCRs display other activation mechanisms. For example, opsins are activated by a photon, and the thrombin receptor activates itself after its N-terminus is cleaved off by thrombin." Quoted from an introduction to GPCRs
An example of a G-protein system for a neurotransmitter receptor:
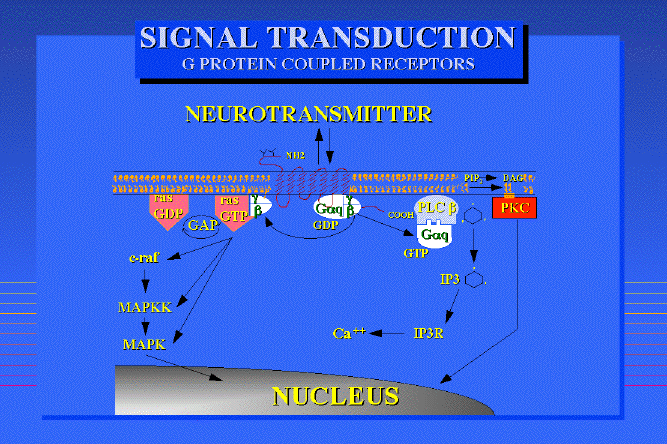
Taken fromDr Philippe Rosay's thesis
Because of the strong interest from the drug industry, much research and structural modeling of the G-protein-coupled receptors has been reported. The GPCR site has links to many models.
Membrane Protein Folding and Stability
General Principles of Membrane Protein Folding and Stability (from the Stephen White Laboratory at UC Irvine)
ATP-dependent transport of ions
Two main families (see Nobel prize for Chemistry, 1997, for brief summary):
- The F1,Fo-family of H+-ATPases (including the vacuolar H+-ATPase).
- The P-type -ATPases (phospho-proteins), with the Na+,K+-ATPase as an example.
Equations for transport, chemical and electrochemical potential gradients
Go back to Lecture 9 for a revision of the equations for transport.
Bacterial membrane transport
Bacterial membrane transport systems allow metabolite transport in (metabolites include a vaste range of small organic molecules, and encompass peptides, nucleic acids, essential metals, etc.), and export of toxins, proteins, pilli, etc. Transport occurs across two membranes. The porins render the outer membrane permeable to small metabolites, but larger molecules require special mechanisms. The cell membrane is imperbeable to all except small uncharged molecules (H2, O2, N2, CO2, NH3, sugars less than 5C) and neutral lipophilic molecules. All ionic metabolites and larger neutral molecules require special mechanisms.
This area has a vaste literature. The Fig. below is a nice summary, in which the molecules with crystallographically defined structures are highlighted. A clickable version, which takes you to the structures is available in R. Bergmann's nice Chime tutorial pages.
©Copyright 1996,
Antony Crofts, University of Illinois at Urbana-Champaign,
a-crofts@uiuc.edu